A Decade of Harvesting Methods
June 1, 2012
The preliminary separation of a protein of interest from a reactor “soup” of process impurities (e.g., cell debris, colloids, lipids) is the first step in a downstream process. It is also a primary step that introduces a significant risk of product degradation, bioburden concerns, or process errors, especially if a harvest method is not a good “fit” with a newly designed bioreactor (e.g., single-use) or fermentation vessel.
In 2003, BPI’s first year, industry concerns revolved around potential capacity shortages, leading manufacturers to seek solutions through enhancing their process operations. One author suggested improving harvesting methods as a solution. “The goal is to recover product cost effectively, regardless of batch-to-batch variability. For maximum transmission of the product, all cells and cell debris must be retained” (1). Today, the concern is not so much about dealing with capacity issues as it is about handling high cell densities from both microbial and cell cultures.
The selected harvesting method and equipment depends on the type of cells, product being harvested, and properties of the process fluids. Traditional techniques include membrane microfiltration, tangential-flow filtration (TFF, or crossflow filtration), centrifugation, and depth filtration, as well as specialized solutions coupling either microfiltration or centrifugation with TFF or depth filtration.
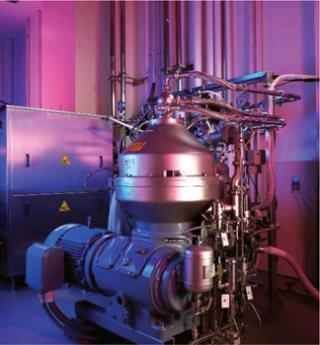
Centrifugation
Disc-stack centrifugation (such as those from Alfa Laval and Westfalia Separator AG) have been part of cell harvesting for some time. A disc-stack centrifuge (pictured above) uses stacked, inclined conical discs to separate out whole cells and large cell debris.
Shearing, however, can damage cells and increase the number of submicron particles that cannot be removed (3). So low-shear systems and techniques coupled with second-stage depth filtration are especially useful (2, 3). The use of depth filtration can provide further clarification, removing smaller solid particulates. Filtration
The bioprocess industry adopted membrane clarification methods from other technologies but has developed modules specific for biopharmaceutical needs, including for primary clarification of fermentation and cell-culture systems. When a membrane technique is used, the size and fouling potential of the membrane are taken into account (1).
Microfiltration is highly sensitive to changes in feedstock quality such as cell culture viability, cell density, and medium components (2). High cell densities and low cell viabilities can result in high transmembrane pressure (TMP) for constant-flux membranes, the extent to which depends on the scale of the feed batch. TMP is relatively lower for typical cross-flow (TFF) microfiltration operations (MF–TFF). The flow of feed solution across the membrane reduces concentration polarization and creates a pressure drop, forcing a size-exclusion condition. Such filters are currently available with various pore sizes, typically between 0.1 and 1 μm for primary harvest (e.g., EMD Millipore’s ProStak system and PallSep Biotech from Pall Corporation).
Depth filtration retains particles that are both larger and smaller than their pore size throughout their porous media. Particle retention is believed to involve both size exclusion and adsorption through hydrophobic, ionic, and other interactions (2). Depth filtration provides some benefits, but it has its limitations (see box). Commercially available technologies include filter sheets (Seitz depth filter sheets from Pall); encapsulated modules (Supracap 200 from Pall); and disposable, scalable formats (e.g., Millistak+ pod depth filter media from EMD Millipore).
Cell Separation Using Depth Filters
Benefits
Low material and hardware costs
Ease of use
Fast process times
High flexibility from scale-up options (e.g., for multiproduct facilities)
Filtration with minimal shearing forces to offer cell and product protection
FDA approval of all materials
High-quality of filtrate
Space savings
Disadvantages
Necessity of monitoring differential pressure
Need to conduct economic evaluation at all scales
Need to remove and dispose of filters
Scale-up limitations (7)
Tangential-Flow Filtration: Methods for harvesting small-scale bioreactors may not work for process scales of 10,000–20,000 L. As Ian Sellick observed, “Handling requirements for such large batches call for employing larger and more continuous types of processing systems, often some form of TFF” (1). TFF is suitable for fine-sized–based separations because it retains particulates and other molecules that are too large to pass through its membrane pores and carries them along with the tangential flow.
TFF configurations include cassettes (flat) and hollow-fiber formats. In his article, Sellick describes a compact TFF cassette device that uses polyethersulphone membranes and screens to form diagonal flow paths that prevent product channeling (which occurs when membrane surface area is not fully used and the feed channels to the retentate). He also describes enhanced hollow-fiber modules for cell perfusion that used a nonbinding polyvinylidene difluoride (PVDF) (1). Disposable, presanitized TFF cassettes provide several benefits over many traditional cassette formats, including cost savings, lower risk or cross contamination, and greater manufacturing flexibility (4).
In another study, Rechtsteiner et al. described a system of lenticular depth filters (as alternative to TFF methods) for the cell separation from a recombinant CHO line (5). Testing scaled from 20 L to 400 L of cell suspensions. Optimization
Efforts have been made to correlate the characteristics of a cell culture (viability, density, for example) and the efficiency of a harvest process. In one study of a high-cell density culture, the quality of the primary recovery depended on cell density and cell viability. High cell densities and low viabilities result in larger numbers of whole cells and solid impurities such as colloids and cell debris. High titers typically generate from conditions of high cell densities and low viability. Using turbidity as a marker for product quality, researchers demonstrated a linear correlation between cell culture viability and clarification efficiency for a given centrifugation condition (3). Their results also showed that higher feed rates lowered clarification because of lower centrifuge residence times and that the correlations for the centrifuge were the same as those shown for the subsequent depth filtration step.
In another study, researchers monitored pressure in a depth filtration process (second step after disc-stack centrifuge) to achieve better process control and maximize processing efficiency (6). Researchers clarified a cell-culture harvest from a 1,000-L reactor and used proprietary disposable pressure sensors (PressureMAT system, PendoTECH) to demonstrate that their approach allowed for “frequent, accurate, and automatic pressure readings that provide a complete picture of depth filter performance” (6).
The increasing use of single-use technologies in upstream processes may require a shift in harvest strategies. According to Pegel, et al: “With traditional fed-batch processes, harvest clarification is usually achieved by centrifugation followed by depth filtration. For processes based entirely on disposables, the disc-stack centrifuge needs to be replaced by filtration alone” (7). Their concept study involved evaluation of various commercially available single-use depth filtration systems for potential implementation into a 1,000-L high– cell-density monoclonal antibody platform process. The article by Minow et al. in the June 2012 issue presented with this supplement provides an example of one application of SU depth filtration in the design and implementation of a fully disposable manufacturing facility for the production of monoclonal antibodies. The article by Shukla in the June 2012 issue discusses other single-use centrifugation options. Scale Challenges
The current trend toward small-scale batch process will necessitate primary recovery techniques that can provide quick turnarounds. Royce described a system of glass-fiber filters coupled to a retentive membrane to provide “a rapid solution that can be implemented with very little preparation” (8). Glass-fiber and polyethersulfone membranes are inherently low in extractables and have been validated for low levels of endotoxin and other contaminants. They are steam sterilizable and compatible with gamma irradiation, enabling preuse sterilization with either method. The solution presented by Royce and his colleagues offered a solution designed to work reliably in the majority of mammalian cell culture applications with no upfront process development efforts.
TFF and c
entrifuge–depth filtration are typical methods for harvesting large-scale batches. But process transfer sometimes requires alternative, streamlined approaches. A Genentech study evaluated various dual-layer, single-stage depth filter media to find an alternative to its traditional two-stage depth filtration train to be used at a separate facility (9). The work involved testing at various turbidities, resulting in a system that demonstrated increased capacity at all levels without significant plugging of the internal pore structure of the depth filters. Researchers further suggest that “one key to increasing future depth-filter capacity may… be to control particulate clustering… or modulate flow rate to avoid or exploit critical shear thresholds within a depth filter” (9).
High-Density Cell Cultures: As titers increased during this past decade, so too did the importance of efficient product recovery methods. With monoclonal antibody titers reaching ∼25 g/L and the expectation of even greater values in the future, clarification of high-density cell cultures will present unique challenges to biomanufacturers. Ever-increasing amounts of cell debris will need to be removed quickly to prevent bottlenecks in downstream processes. Possible solutions could include perfusion-derived systems (e.g., ATF system from Refine Technology) in which both product and cells are temporary retained in suspension culture.Scientists at Percivia have developed such a system (eXtreme Density, XD, cell culture process), which retains product inside a bioreactor. Ion-exchange (IEX) matrices are then used to induce “cell settling” in situ to generate a partially clarified supernatant. A two-stage filter train (from EMD Millipore and Cuno) completes depth filtration. According to their study, the system “enhances cell settling and offers an effective alternative to centrifugation for clarifying XD PER.C6 (Crucell Holland BV Corporation) cell culture harvests” (10).
About the Author
Author Details
Maribel Rios is managing editor of BioProcess International;[email protected].
REFERENCES
1.) Sellick, I. 2003. Improve Product Recovery during Cell Harvesting. BioProcess Int. 1:62-65.
2.) Roush, DJ, and Y. Lu. 2008. Advances in Primary Recovery. Biotechnol. Prog. 24:488-495.
3.) Iammarino, M. 2007. Impact of Cell Density and Viability on Primary Clarification of Mammalian Cell Broth: An Analysis Using Disc-Stack Centrifugation and Charged Depth Filtration. BioProcess Int. 5:38-50.
4.) Chochois, H, J Blehaut, and M. Labreck. 2009. A Presanitized, Purpose-Designed, Single-Use TFF Strategy. BioProcess Int. 7:S36-S40.
5.) Rechtsteiner, H. 2004. Cell Separation from Mammalian Suspension Cultures. BioProcess Int. 2:60-62.
6.) Bink, LR, and J. Furey. 2010. Using In-Line Disposable Pressure Sensors to Evaluate Depth Filter Performance. BioProcess Int. 8:44-49.
7.) Pegel, A. 2011. Evaluating Disposable Depth Filtration Platforms for MAb Harvest Clarification. BioProcess Int. 9:52-56.
8.) Royce, J. 2009. Development of a Turn-Key Harvest Solution for Small-Volume Bioreactors. BioProcess Int. 7:24-30.
9.) Dave, P, J Dizon-Maspat, and T. Cano. 2009. Evaluation and Implementation of a Single-Stage Multimedia Harvest Depth Filter for a Large-Scale Antibody Process. BioProcess Int. 7:S8-S17.
10.) Schirmer, EB. 2010. Primary Clarification of Very High-Density Cell Culture Harvests By Enhanced Cell Settling. BioProcess Int. 8:32-39.
You May Also Like