Linear Scale-Up of Cell Cultures
Reusable bioreactors have been the benchmark standard for many decades, during which a large knowledge base on process control and scale-up has been developed. However, single-use bioreactors are increasingly being implemented in modern bioindustrial upstream processes. Many of these bioreactors deviate from the traditional stirred-tank design, but a number of companies have expressed a strong need for single-use bioreactors based on the strirred-tank design. A traditional stirred-tank design would enable users to optimize their scale-up processes based on both experience and well-established guidelines regarding scale-up elements as tip speed, mixing time, oxygen transfer, and specific power input. To meet that demand for improved scalability using single-use bioreactors, New Brunswick Scientific (NBS) has developed a new stirred single-use bioreactor called the CelliGen BLU system.
Single-Use Benefits
A stainless-steel bioreactor has predefined, fixed vessel port assemblies on both its headplate and vessel body. Because the culture chambers of disposable bioreactors are constructed from presterilized plastic material, their number, size, and location of their port assemblies can be easily and quickly altered and made at relatively little cost. That makes single-use cultivation vessels highly convenient to specific process applications. Disposables are presterilized by their suppliers, eliminating user cleaning and sterilization between runs — which in turn eliminates expensive capital investment in equipment for clean-in-place (CIP) and steam-in-place (SIP) operations. It reduces production turnaround times between runs and the costs and time associated with validation of SIP/CIP. Less power, water for injection (WFI), and labor are needed, and downtime associated with stainless equipment, labor, CIP, and SIP is no longer an issue. The risk for cross contamination is also eliminated. These benefits facilitate operation of multiple-product facilities and increase product output. Ultimately, application of single-use systems shortens time to market and provides a higher and faster return on investment (ROI).
PRODUCT FOCUS: ALL BIOLOGICALS
PROCESS FOCUS: PRODUCTION
WHO SHOULD READ: PROCESS AND CELL CULTURE ENGINEERS, PRODUCT DEVELOPMENT, MANUFACTURING
KEYWORDS: BIOREACTORS, SINGLE-USE TECHNOLOGY, STIRRED TANK, IMPELLERS, SCALE-UP, SENSORS, CONTROLLERS
LEVEL: BASIC
We compared the time required for a prerun set-up, cultivation, and postrun clean-up using an autoclavable 5-L glass vessel with the time required for a single-use cell culture run in a CelliGen BLU system. Table 1 details the significant time savings of nine hours using the disposable bioreactor.
Table 1: Comparing set-up and clean-up times for reusable glass vessel and CelliGen BLU bioreactors
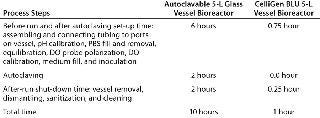
Table 1: Comparing set-up and clean-up times for reusable glass vessel and CelliGen BLU bioreactors ()
The growing number of single-use bioreactor suppliers has helped these technologies rapidly mature, leading to the availability of a wide range of systems that provide for high cell densities and product titers (1). However, some difficulties remain to be overcome before disposable bioreactors can make a fully successful transition to commercial manufacturing processes. Reusable stainless-steel bioreactors are clearly characterized in terms of their performance, optimization steps, and design. They have been the benchmark for many decades and are supported by a large amount of knowledge and experience on process control on the operator side. Use of disposable bioreactors based on a stirred-tank design could provide for more safety, scalability, and robustness to bioprocessing.
Stirred-Tank Advantages
Scalability is an essential element in all manufacturing processes. Developers of industrial cell culture processes for manufacture of recombinant biologics seek high efficiency, reproducibility, and predictability. Typically, the time allotted for scale-up protocols and process development to achieve defined culture conditions is short enough to maximize cell productivity and yield while minimizing process scope and overall cost. Having identically designed bioreactors throughout a complete manufacturing process significantly facilitates scale-up.
Hybrid solutions are becoming increasingly popular in manufacturing processes for which stainless-steel bioreactors are used at larger volumes (50–3,000 L) and multiple single-use bioreactors at 5–100 L. In scaling-up, culture broth from a single-use bioreactor is transferred into a larger-scale stainless-steel bioreactor. This suggests a clear need for single-use bioreactors with a stirred-tank design and setup that would be comparable to the larger stainless steel reusable alternatives. The more unfamiliar a technology, the more questions both users and regulators will have about it. Even though stirred-tank, single-use bioreactors are widely used for many cell culture processes, most are limited when it comes to the need for comparability during scale-up. Some examples include eccentric stirrer motions, nonvertically installed stirrers, and deviations from standard impeller and sparger types.
Stirred-tank bioreactors are the most commonly used reusable bioreactors for culturing suspension cells, mainly because of the broad experience that’s been obtained in microbial fermentation using the same type of culture vessels. Basic design criteria have been derived from those of microbial cultures and modified to meet the requirements of mammalian cells. Specifically, the shear sensitivity of animal cells requires consideration in impeller design, aspect ratio, and oxygenation. Scale-up principles for these reactors are generally better characterized than are those of other bioreactor types. The stirred-tank design is already a well accepted technology with regulators for manufacturing biologics and other drugs. And it is a predictable technology in terms of process scale-up.
Keeping in mind those benefits, New Brunswick Scientific developed its single-use, rigid-walled, stirred-tank CelliGen BLU bioreactors (Figure 1), which are currently available in 5-L and 14-L interchangeable sizes. The company’s focus in developing this bioreactor was to improve comparability of single-use and classical processes by adopting a stirred-tank design. To that end, several critical design criteria were taken into account.
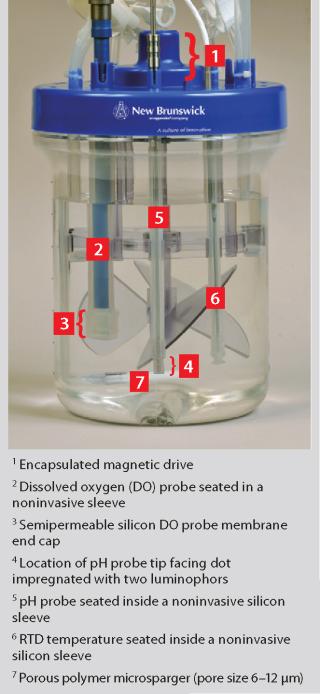
Figure 1: ()
Vessel Design: The height/diameter ratio of Celli
Gen BLU vessels is within the limits (as listed in the “Design Parameters” box) of typical stirred-tank vessels for cell culture applications — which range 1:1–2.3:1 — and they have a maximum working volume of 70–80%. The relatively high surface/volume ratio and the higher residency times of gas bubbles from direct sparging (by the porous microsparger) improve gas transfer rates, enabling medium oxygenation in the headspace. In disposable bag-type bioreactors, an outer container or holder is needed to ensure the integrity of bag-design characteristics during process runs. The vessel is made of a clear, rigid plastic that eliminates the need for a supporting outer container. The rigid design also circumvents severe folding stresses that are likely to occur in the plastic film of bags under internal medium pressure, which may lead to cracks and leaks. NBS is considering broadening the current volume range of the CelliGen BLU sysems to a cover range from lower milliliters up to tens of liters.
Agitation in stirred-tank bioreactors is intended to provide homogeneous suspension of cells and prevent chemical (nutrients and waste products), physical (pH, oxygen, and carbon dioxide), and thermal gradients inside the vessels. Large impellers with axial fluid-flow characteristics (e.g., pitched-blade impeller design) are typically used to minimize shear rates. CelliGen BLU vessels use an impeller with three pitched blades capable of up-flow or down-flow axial mixing. The blades are pitched at a 45°angle to their horizontal axis, with an impeller-to-vessel diameter ratio of ~0.5, which correlates to the classical ratio of 0.3–0.5 to allow efficient mixing with the low shear force. The power number (Np) of the impeller is 1.3. NBS may soon evaluate incorporating its proprietary packed-bed basket impeller into CelliGen BLU vessels for high–cell-density culture of anchorage-dependent or secreting suspension cell lines.
CELLIGEN BLU VESSEL DESIGN PARAMETERS
Ratio of vessel diameter to impeller diameter (5-L vessel): 1.7:1.0
Ratio of vessel diameter to impeller diameter, 14L vessel: 2.1:1.0
Ratio of vessel height to diameter (5-L vessel): 1.5:1.0
Ratio of vessel height to diameter (14-L vessel): 2.0:1.0
Gas flow rates (overlay): 0.1–3.0 SLPM
Gas flow rates (through sparger): 0.002–0.100 SLPM (total gas flow rate through sparger per TMFC)
Aeration and Gas Control Loops: Curtailing mechanical forces is not the only design criterion important to oxygenation. Sufficient oxygen (O2) transfer and carbon dioxide (CO2) removal are also important when selecting a bioreactor system. Oxygen is a key nutrient in cellular metabolism. Even though related demands of animal cell cultures are several orders of magnitude lower than are those of microbial cultures, oxygenation is often the primary challenge in cell culture scale-up. The importance of aeration increases with bioreactor volume and cell concentration. Whereas low oxygen concentrations negatively affect cell growth and yield, excessive oxygen tensions can be toxic to cells.
The classical and most widely used aeration method is direct sparging: bubbling gas directly into the culture medium. Proper sparger selection is important for minimizing cell damage and foaming while increasing aeration. Air or oxygen is sparged into animal cell cultures with ring spargers similarly designed to those used in microbial fermentors or with microspargers made of sintered metal or polymeric materials.
Microspargers provide for significantly higher gas–medium interface and thus higher O2 mass-transfer coefficients with the same gas flow rate, which effectively reduces the overall superficial gas flow rate. But despite their mass transfer efficiency, very small bubbles (
Even when other aeration methods are used, flushing air through the headspace compartment of a bioreactor provides an additional means to increase overall OTR and remove CO2. To meet higher demands for oxygen by cells in culture, one option is to increase the gas flow rate flowing through the sparger. But too high a gas flow rate will cause bubbles to coalesce, which decreases the gas–liquid interface and increases shear.
To address those problems, the CelliGen BLU system is equipped with two independent, completely flexible gassing systems that automatically maintain dissolved oxygen (DO) and pH setpoints. Both are automated using a proportional–integral–derivative (PID) controller. The first gassing system is for direct gassing through the vessel sparger, and the second is for flushing the headspace. In both systems, up to four gases (air, O2, N2, and CO2) can be automatically proportioned in correct amounts by a bank of four solenoid valves, depending on feedback coming from the DO and pH sensors. The gas mixture then passes through a thermal mass flow controller (TMFC) to the sparger and/or vessel headspace. The TMFC controls the overall flow rate of a gas mixture set by the user. The CelliGen BLU system can also have three or four TMFCs, which negate use of the four solenoid valves.
For animal cell cultivation, total gas flow rate through the sparger depends on the type used. For a ring sparger, generally the total gas flow rate is between 0.1–0.3 vvm (gas volume flow per unit of liquid volume per minute). In the CelliGen BLU system, where a microsparger is used, the total gas flow rate is 0.007–0.03 vvm. A maximum gas flow rate of 0.03 vvm — 0.1 standard liters per minute (SLPM) — through the microsparger meets the generally recommended flow rate for cell cultures. This flexibility in aeration gives operators complete control of their cultivations and enables them to change processes without problems either during cultivation or between applications.
Single-Use Magnetic Drive and Noninvasive pH and DO Probe Sleeve Design: The CelliGen BLU vessel headplate has a unique, single-use magnetic drive design comparable to the magnetic drive of reusable stainless-steel vessel headplates. The magnet is completely sealed and encapsulated by a polycarbonate headplate (Figure 1), with the impeller shaft drive bearing attached to it. The impeller drive shaft is held in place by two high-density polymer drive bushes (sleeves), which provide lateral stability.
Figure 1 shows the innovative, noninvasive pH sensor sleeve and DO probe silicone polymer sleeve with its unique gas-permeable end cap. The silicone end cap at the tip of the DO sleeve allows oxygen to readily diffuse from the medium through the silicone end cap into the DO probe tip, where it is detected. This design has several advantages over the methodology of inserting autoclaved DO probes. The silicone end-cap membrane design allows air to escape when a DO probe is inserted into its sleeve. Once that is fully inserted, and the probe tip is in contact with the silicone end-cap membrane, the DO probe tip is sealed off air-tight, eliminating interference from the air. The noninvasive sleeve completely eliminates contamination risks because there is no contact between the vessel interior and the space within the DO sleeve. This saves a significant amount of vessel set-up time (several hours) by eliminating repeat polarizations of the DO probe for each run. It allows use of a nonsterile, conventional polarographic DO probe, eliminating repeat autoclaving of the DO probe and hence extending its life. The pH probe sleeve is also noninvasive
as part of a special optical sensing system manufactured by PreSens Precision Sensing, GmbH (www.presens.de).
The Presens device consists of a metal probe connected to the controller at one end. The other end of the probe periodically emits a fluorescence light (excitation wavelength) that excites two luminophors impregnated onto a dot adhered to the bottom of the noninvasive silicone sleeve. The pH sensor’s principle of operation is conversion of the luminous intensities (emitted light) of the two luminophors — one a pH-sensitive indicator and the other a reference indicator — into a pH value. Both luminophors are excited by light emitted from the probe tip, and they fluoresce at a different wavelength from its source. The luminophors have different response times so that when the excitation light’s intensity is sinusoidally modulated, the phase shift between the source and the combined response of the two luminophors is calibrated to a pH value.
The reusable pH sensor probe device comes with the CelliGen BLU control cabinet. Unlike a traditional glass gel-filled pH probe, it is very easy to use: Simply insert a probe into the noninvasive sleeve to eliminate both autoclaving and maintenance care required for glass gel-filled pH probes.
New Biopharmaceutical Market Demands
The biopharmaceutical Industry is moving rapidly to adopt single-use bioreactors. Indeed they are being used in commercial production now for inoculum seed trains at small scale. But stirred, single-use bioreactors face some barriers to large-scale manufacturing. Many low-potency, high-dose biopharmaceuticals are produced at large scales (10,000–20,000 L) to meet commercial quantities.
Nonetheless, market trends on new drug manufacturing show that new biologics drugs emerging through the pipeline are of considerably higher potency than those already on the market. In addition, productivity is increasing for these high-efficacy drugs (the amount of drug produced from each cell in a bioreactor), which allows smaller dose volumes to be used. Consequently, manufacturing volumes are also significantly smaller.
Markets are also a shrinking: Some drugs are specifically designed for narrower patient populations and tailored to specific patient responders and receptors (e.g., the receptor-based clinical diagnostic drugs administered to patients who show evidence of having specific receptors for a given drug). The main feature of this high-potency, small-dose drug niche market is that it will be small, with large numbers of different drugs produced in much smaller batch sizes than current lots — with several different products made in each facility.
The challenge for biologics manufacturers will be how to lower the overall cost of making such drugs while generating a good return on investment. Stirred, single-use bioreactors in 5-L to 2,000–L sizes can provide a cost-effective solution to meet both development and the commercial demands for niche-market drugs. We believe increasing percentages of marketed drugs will be produced using stirred, single-use bioreactors of 1,000-L to 2,000-L sizes. Further, the size of such bioreactors required for R&D, preclinical, and clinical work pertaining to those drugs will be much smaller: in the range of 5–200 L.
Stirred, single-use bioreactors can bring significant improvements to animal cell cultivation processes. Like other single-use bioreactors, they can make cell culture operations more flexible, cheaper, and less time consuming. And thanks to their correlation with the classical design of reusable bioreactors, these bioreactors can transcend existing challenges. They represent an important step toward the complete maturity of single-use technologies. Stirred, single-use bioreactors can help create a more modern, efficient, flexible, and multipurpose biopharmaceutical production facility.
About the Author
Author Details
Corresponding author Vikram Gossain, PhD, s applications and training manager, and Rich Mirro is a product manager at New Brunswick Scientific, PO Box 4005, Edison, NJ 08818-4005; 1-732-650-2012, fax 1-732-287-4222; [email protected]; www.nbsc.com.
REFERENCES
1.) Scott, C. 2007. Single-Use Bioreactors: A Brief Review of Current Technology. BioProcess Int. 5:S44-S51.
2.) De Wilde, D. 2009. Bridging the Gap from Reusable to Single-Use Manufacturing with Stirred, Single-Use Bioreactors. BioProcess Int. 7:S36-S40.
3.) Eibl, R, and D. Eibl. 2009. Disposable Bioreactors in Cell Culture–Based Upstream Processing. BioProcess Int. 7:S18-S23.
4.) Feliza, M. 2008. Disposable Bioreactor Use Grows in Commercial Production: A Disposable Future. ICIS Chem. Business:26-28.
5.) Fuller, M, and H. Pora. 2008. Introducing Disposable Systems into Biomanufacturing: A CMO Study. BioProcess Int. 6:30-36.
6.) Mirro, R, and K. Voll. 2009. Which Impeller Is Right for Your Cell Line?. BioProcess Int. 7:52-57.
7.) Pais-Chanfrau, JM, K Zorrilla, and E. Chico. 2009. The Impact of Disposables in Project Economics in a New Antibody Plant: A Case Study. BioPharm Int.:62-74.
8.) Integration of Disposable Technology, One Company’s Perspective 2008.. BioProcess Int. 6:S42-S46.
9.) Vogt, R, and T. Paust. 2009. Disposable Factory or Tailor-Made Integration of Single-Use Systems?. BioProcess Int. 7:S72-S77.
You May Also Like