A Review of Therapeutic Protein Expression By Mammalian Cells
During the past five years, many biopharmaceuticals have found their way into clinical trials and commercial production (1–4). So far, about 60 million patients worldwide have benefited from these new drugs. The market for biopharmaceuticals was estimated at US$33 billion in 2004 and projected to reach US$70 billion by the end of the decade. During the period 2003–2006, regulators in Europe and the United States approved 32 biopharmaceuticals for human use, including hormones and growth factors, therapeutic enzymes, vaccines, and monoclonal antibody (MAb)-based products. An additional 1,600 biopharmaceuticals are being evaluated in clinical trials.
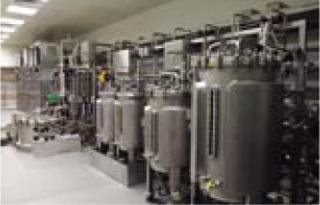
Figure 1: Addition vessels for a GMP 1500-L fermenter at SynCo BioPartners, a biopharmaceutical contract manufactuerer in The Netherlands ()
MAbs constitute by far the largest product category, with the number of such product candidates rising from 75 to 400 in the period of 2003–2006. The global sale of MAbs in 2006 were US$20.6 billion (5–8), and currently 25 are approved for the market. It is expected that many new MAbs will be approved within the coming years. As Figure 1 shows, demand is rapidly increasing, making MAb-based products the most rapidly growing category of biopharmaceuticals (6).
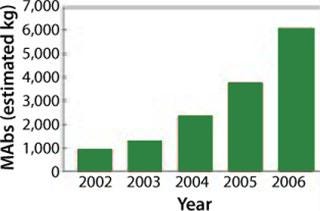
Figure 1: ()
That increase has been enabled by improvements made in the recombinant expression technologies that use mammalian cells — particularly as MAb production went from mouse-based systems through chimeric to fully human antibodies. Mammalian expression systems provide a basis for producing large quantities of biopharmaceutical proteins. These systems are gaining market share because they are becoming better understood and thus offer improved performance in yield, speed, and quality (Figure 2). However, significant improvements are still needed to handle the increasing demand for MAbs.
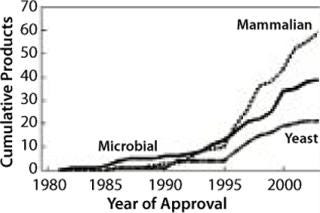
Figure 2: ()
Mammalian Cell Lines
The most used mammalian cell lines for biopharmaceutical production are Chinese hamster ovary (CHO), myeloma, and hybridoma cells. Considerable progress has been made in optimizing these systems, particularly the NS0 myeloma and CHO cell lines. Both expression systems are currently predominant in biopharmaceutical protein production. Fine-tuning of vector construction, identification and use of selectable markers, optimization of transfection in production media, gene targeting, and high-throughput screening have led to high specific productivities ranging 20–60 pg × cell−1 × day−1 (9–12). That is in most cases accomplished by gene amplification, which makes cells more prone to instability (13–16). Also, lead times for clone generation can be shortened to 3–5 months for certain clones.
Other mammalian cell lines — e.g., human embryonic kidney (HEK) 293, baby hamster kidney (BHK), and Cos cells — are used to a lesser extent, mainly for virus and vaccine production (17–19). The “Cell Lines” box details those most common. All cell lines used for biopharmaceutical protein production until now originate from mammals. However, certain cell lines have recently been derived from duck embryonic stem cells for use in vaccine production, replacing many old vaccine production processes based on embryonated eggs. Such nontumorigenic cell lines grow in suspension on serum-free media and have additional advantages: a lack of endogenous retroviruses and no risk of cross contamination with human adventitious agents (20). They can not only be used for expression of therapeutic proteins, but also have a significant potential for vaccine manufacture. Vaccine development using these novel cell lines from nonmammalian origin will probably replace the egg-based manufacturing in the near future.
Most biopharmaceuticals on the market are produced in CHO-K1 or CHO-derived cell lines such as DHFR-, DG44, and DUK-B11 cells. These have been used extensively for high-level protein production because they enable amplification of specific genes through selection with an amplifiable marker such as dihydrofolate reductase (DHFR) or glutamine synthetase (GS). GS can act as a dominant selectable marker, which can theoretically be used in cells that express endogenous GS as well as those that do not. A number of proteins have been expressed in CHO cells: enzymes; recombinant MAbs; blood coagulation factors; and other cell surface markers such as chemokines, adhesion molecules, growth factors, and their receptors (21–24).
Myeloma cell lines — e.g., NS0, Sp2/0, and P3X63.Ag8.653 — have also been used commonly for high-level MAb production. NS0 mouse myeloma cells are often favored as a production cell line because they contain very low levels of endogenous GS compared with CHO cells. It is assumed that the high productivity of the NS0-GS system comes from the fact that the cells are derived from immunoglobulin-producing tumor cells, which have the innate machinery for antibody production. Another important feature of these myeloma cells is their tendency to grow well in suspension when adapted to serum-free media. Several products are already on the market using this expression system. It must be mentioned, however, that instability as well as relatively slow growth have been reported (13).
A novel mammalian expression system currently under evaluation by several biopharmaceutical companies uses PER.C6 technology from Crucell Holland BV (www.crucell.com) in the Netherlands, which is based on a human cell line (25, 26). This expression platform offers significant advantages over the established systems mentioned above. It requires no amplification of inserted genes to deliver stable clones with high levels o
f protein expression in several months. Nor does it require any selection agent, as has been used by the DHFR-system. A low copy number is sufficient to retain stable and efficient protein expression. Initial results showed a significant potential for MAb and recombinant protein production. Productivities >2 g/L could be reached in fed-batch cultures, 0.9 g × L−1 × day−1 in continuous perfusion cultures, with peak productivities up to 3 g × L−1 × day−1 without compromising product quality (25–27). Because of their human origin, PER.C6 cells do not synthesize potentially immunogenic MAb glycan structures. Productivities of 0.9 g × L−1 × day−1 in homogenous continuous perfusion systems are considered a breakthrough. Corresponding viable cell densities are around 100 × 106 cells ×mL−1, with peak cell densities of 150 × 106 cells × mL−1 (28). To our knowledge, those numbers have never been reported before. Such achievements suggest a future of small and extremely high-producing mammalian cell culture systems.
Improvements
Over the past few years, improvements in mammalian expression systems have focused on genetic modifications of host cells for improving their ability to produce more recombinant proteins with the proper posttranslational modifications (for product quality). Many studies have focused on metabolic control of cell growth: regulating life cycles and limiting apoptotic death.
Production processes can be improved by regulation of proliferation after allowing cells to grow rapidly at first and achieve a sufficiently high cell density for production. That growth period is followed by a production phase, in which cell density is maintained at the same level by inhibiting apoptotic cell death. Cyclin-dependent kinases (CDKs) are good targets for such control of proliferation because that particular family of protein kinases plays a crucial role in processes that control cell cycle transitions. Their activity is regulated by binding CDKs with cyclins to form complexes (after phosphorylation) with cyclin-dependent inhibitors in the CIP/Kip family, such as p21, p27, and p57 (3, 29, 30).
MENTIONED CELL LINES
CHO: an epithelial cell line derived from the ovaries of Chinese hamsters (Cricetulus griseus)
DHFR−: a CHO cell line based on CHO duk cells (dhfr refers to dihydrofolate reductase); for example, see ATCC* catalog number CRL-9096
DG44: a CHO cell line
DUK-B11: a CHO cell line
NS0: a myeloma cell line derived from B lymphocytes of mice (Mus musculus); for examples, see ATCC* catalog numbers CRL-1827, -2695, and -2696
HEK293: an epithelial cell line derived from human embryonic kidney cells transformed with adenovirus 5 DNA; for example, see ATCC* catalog number CRL-1573
BHK: a cell line derived from the kidney cells of baby Syrian golden hamsters (Mesocricetus auratus); for example, see ATCC* catalog number CRL-1632
COS-1, COS-7: fibroblast cell lines derived from the kidney cells (SV40 transformed) of African green monkeys (Cercopithecus aethiops); for examples, see ATCC* catalog numbers CRL-1650 and -1651
Sp2/0: a lymphoblast myeloma cell line derived from B lymphocyte hybridomas of mice (Mus musculus); for example, see ATCC* catalog number CRL-2016
P3X63.Ag8.653: a lymphoblast myeloma cell line derived from B lymphocytes hybridomas of mice (Mus musculus) and rats (Rattus norvegicus)
PER.C6: a trademarked cell line (derived from a human retinal cell) developed and owned by Crucell Holland BV
*www.atcc.org/SearchCatalogs/CellBiology.cfm
In several studies, p21cip and p27kip were overexpressed to cause cell cycle arrest at the G1 phase of the cell cycle. Productivity of recombinant proteins increased as a consequence. Al-Rubeai et al. showed a fourfold increase in productivity by p21cip overexpression resulting from a lengthening of the cell cycle, which increased mitochondrial mass and activity and improved ribosome biogenesis (31).
Programmed cell death (apoptosis) occurs in essentially all industrially available cell lines: e.g., CHO, myelomas, hybridomas, and HEK293 cells. Apoptosis is an obstacle to these cells surviving high cell densities. Therefore its prevention should at least contribute to maximizing productivity of mammalian cells in bioreactors. One of the most investigated pathways involves the Bcl-2 family of proteins. It has both antiapoptotic and proapoptotic members, many of which are associated with mitochondria. Antiapoptotic proteins include Bcl-2 and Bcl-xl, which are localized in the mitochondrial membrane, where they prevent release of cyt c, a cytotoxic protein. A member of the proapoptotic subfamily is Bax, which is also involved in the pathway associated with cyt c release. Several studies have shown that overexpression of Bcl-2 delayed apoptosis during cell culture, consequently improving productivity. Other studies, however, showed only a delay in apoptosis but no productivity increase (32–37). It can thus be concluded that inhibiting apoptosis — whether through the Bcl-2 method or simply by limiting cell culture nutrients — will enhance cellular viability but will not necessarily increase productivity.
DSM AND CRUCELL ANNOUNCE PER.C6 MILESTONE
On 11 March 2008, DSM Biologics of Parsippany, NJ (www.dsmpharmaceuticals.com) and Dutch biotechnology company Crucell Holland BV (www.crucell.com) announced that another important milestone has been achieved with the PER.C6 technology platform for production of monoclonal antibodies and other recombinant proteins. Scientists working at the Percivia Development Center (www.percivia.com) reached a record level titer of 15 g/L at harvest for an antibody product. The development center is a joint venture between DSM and Crucell located in Cambridge, MA.
Percivia CEO Marco Cacciuttolo said, “This high titer achievement — the highest reported to date — marks a major event for the biopharmaceutical industry. It demonstrates the power and robustness of the PER.C6 manufacturing platform, and it clearly shows that this manufacturing platform will have a major impact on the overall economics of manufacturing biopharmaceuticals. DSM and Crucell recognized the enormous potential of this technology when starting the Percivia joint venture just over a year ago. These breakthrough results achieved in a single year reinforce the special properties and abilities of this manufacturing platform. Based on our scientific data today, we are confident that we will be able to drive productivity even further.”
Crucell’s vice president of business development Martina Molsbergen added “The PER.C6 platform can reduce the risk of supply limitations and potentially postpone major capital expenditure decision hurdles for drug development companies.”
Other methods of increasing titers focus on increasing transgene expression levels. Recent improvements include incorporation into transgene constructs of DNA elements that can either act as enhancers or prevent transgene silencing once it has been integrated into a genome. Examples include STAR technology (stimulating and antirepressor elements) from Crucell and other types of chromatin-opening elements, DNA elements used to prevent expression silencing by integration of the constructs into heterochromatin. That
could improve stability — and possibly productivity — while reducing the number of clones to be screened (38–40). The use of artificial chromosomes (e.g., the ACE system from Chromos Molecular Systems Inc., www.chromos. com, of Canada) that function as stable, nonintegrating vectors with large carrying capacity, allowing multiple copies of the same transgene, have resulted in a fourfold higher expression level over CHO-dhfr expression (41). Transgene expression can also be increased by enhancing transgene promoter strength using engineered zinc-finger protein transcription factors that bind a DNA sequence within a promoter (42).
Targeted knockout of mammalian host-cell genes is now possible using RNA interference or engineered zinc fingers (43, 44). Using such an approach, an increase in antibody potency — rather than yield — was obtained by modifying the glycosylation machinery using RNA interference targeting a fucosyltransferase. MAbs produced by such cells have a reduced fucosylation, with a concomitant increase in a particular effector function that is often important for clinical efficacy: antibody-dependent cellular cytotoxicity (45).
Choice of expression system
Process development for biopharmaceuticals is dictated by several key success factors: product quality and safety, speed of generating clinical phase 1–3 material, economy of manufacturing processes for marketed products, and regulatory acceptance. Not surprisingly, those factors also play a key role in evaluating mammalian cell expression systems for use in production of pharmacologically active glycoproteins. To date, the most likely candidates for efficient expression of glycoproteins are mammalian cell lines, especially CHO and mouse myeloma cells.
So the most important factors to take into consideration when choosing an optimal expression system, are productivity, process economics, product quality and safety, lead times, scalability, and regulatory acceptance.
Productivity: For glycoproteins, expression levels are mainly determined by the promoter construct, its copy number and site of integration into the chromosome, and the type of protein in question. Volumetric productivity (VP) is determined by the specific productivity and growth performance of cells. It will ultimately determine the total yield of an expression system/process. Both specific productivity and maximum cell density directly affect the required culture volume and production scale for a given amount of MAbs.
Table 1 provides an example in which VP is mainly determined by the maximum cell density reached in a continuous, steady-state perfusion culture. Intrinsic productivity is 15 pg × cell−1 × day−1 and, in one case, the maximum cell density is 10 × 106 cells × ml–1. In the other case, 100 × 106 cells × mL−1 is reached using the PER.C6 human cell line. As a result, VP is tenfold higher in the high–cell-density culture. A required amount of 10 kg purified protein can be produced in 11 runs at the 500-L scale using the lower-density cultures — compared with only one run in a high-productivity culture. In addition, a tenfold smaller amount of cell culture medium is required.
Table 1: Comparing high- and low-producing cell lines for production of 10 kG of recombinant proteins in a continuous perfusion culture
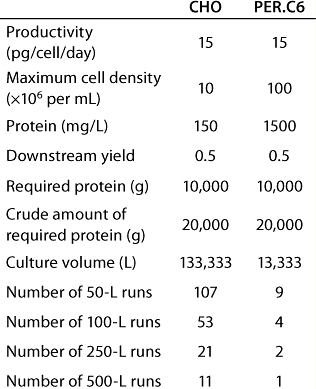
Table 1: Co
Figure 3 shows the same relationship in achieving 10 kg of product. Again, at lower VPs, more runs are needed to obtain the same amount of product. The examples show that improving specific productivities in combination with high cell densities will significantly affect process economics: costs of goods and use of plant capacity. Recent data show current MAb productivities at the level of multiple grams per liter have been achieved in CHO, NS0, and PER.C6 cell lines (28).
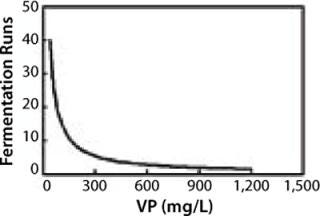
Figure 3: ()
Product quality is predominantly determined by posttranslational modifications, including glycosylation and protein folding, which will largely determine a molecule’s biological activity. Differences among the mammalian expression systems are well documented, particularly for CHO and NS0 cells (46). Despite those differences, most approved MAbs are produced in one or the other system.
Glycosylation patterns and protein structures are influenced by host-cell enzymatic systems as well as bioreactor conditions (24, 47). Therefore, selection of host cells and culture conditions must take into account the requirement for stably producing each specific glycosylation pattern. So appropriate analytical tools must be used for in-process monitoring to determine early in a cell culture process, whether changes due to culture conditions might occur. Several protein analytical tools are available for assessment of glycovariants: peptide mapping, isoelectric focusing, oligosaccharide mapping, matrix-assisted laser desorption ionization time of flight (MALDI-TOF) mass spectrometry, capillary electrophoresis, and specific potency assays.
Works in Progress
Several mammalian expression platforms have been investigated during the past decade. Most MAb products are produced using CHO- and NS0-based systems. Novel technologies are being explored, from improving DNA vector constructs to optimizing existing cell lines as well as novel platforms. Such technologies hold great promise. For example, the PER.C6 human cell line has already outperformed established systems and brought new insights in mammalian cell technology (see the “Milestone” box).
In addition to yield improvements, efforts are being made to enhance cellular properties that enable more efficient and effective process performance. One continuing goal is for smaller scale processes to better predict large-scale performance in industrial settings. Improvements discussed here should lead to cost-effective production of biopharmaceutical proteins for treatment of life threatening diseases.
References Online
Find all the references (1–47) for this review article online at www.bioprocessintl.com.
You May Also Like